Learning and Memory
Video – Author/Source: Ted Talks; The difference between classical and operant conditioning – Peggy Andover
Why is it that humans react to stimuli with certain behaviors? Can behaviors change in response to consequences? Peggy Andover explains how the brain can associate unrelated stimuli and responses, proved by Ivan Pavlov’s famous 1890 experiments, and how reinforcement and punishment can result in changed behavior.
Video – Author/Source: Neuron Theory M.D.; Henry Molaison: How Patient HM Changed What We Know About Memory
In 1953, Henry Molaison (known in the academic literature simply as “Patient HM”) agreed to undergo an experimental procedure where a part of his brain located in the medial temporal lobe was removed. He woke up from the lobotomy unable to form any new memories. Scientists were puzzled as they realised that any new memories Molaison had acquired before the surgery remained largely intact. Eventually, Henry Molaison became the most studied patient in the history of psychology, becoming the subject of endless research papers and books.
Reading – Quiñones et al., 2020 – Am K Alzheimers Dis Other Demen – An Agenda for Addressing Multimorbidity and Racial and Ethnic Disparities in Alzheimer’s Disease and Related Dementia
Reflection Reading #5 – Chen, Ding, & Wang, 2023 – Nature Medicine – Digital health for aging populations
Chapter 13: Learning and Memory
Think back to your favorite birthday party. Which of your friends were there? What did you do, where did you go, and did you have cake? Did you get gifts?
The ability to perform this task depends on our ability to create and recall memories. According to our current best understanding of the neuroscience of learning, the underlying biology of a memory mainly consists of subtle changes among synapses distributed across several brain areas. Our ability to learn new facts, recount the events of last week, or to perform new motor skills is the result of learning-induced neural plasticity. In this chapter, we will consider different aspects of learning and memory, starting from the behavioral level down to the molecular changes responsible for memory formation, as well as some disorders that disrupt healthy memory processes.
13.1 Patient HM
One of the most influential case studies in the neuroscience of memory is the story of Patient HM. HM was born in 1926 in a small Connecticut town. He had a mostly regular childhood: taking family road trips, riding bicycles, and learning about American presidents in school.
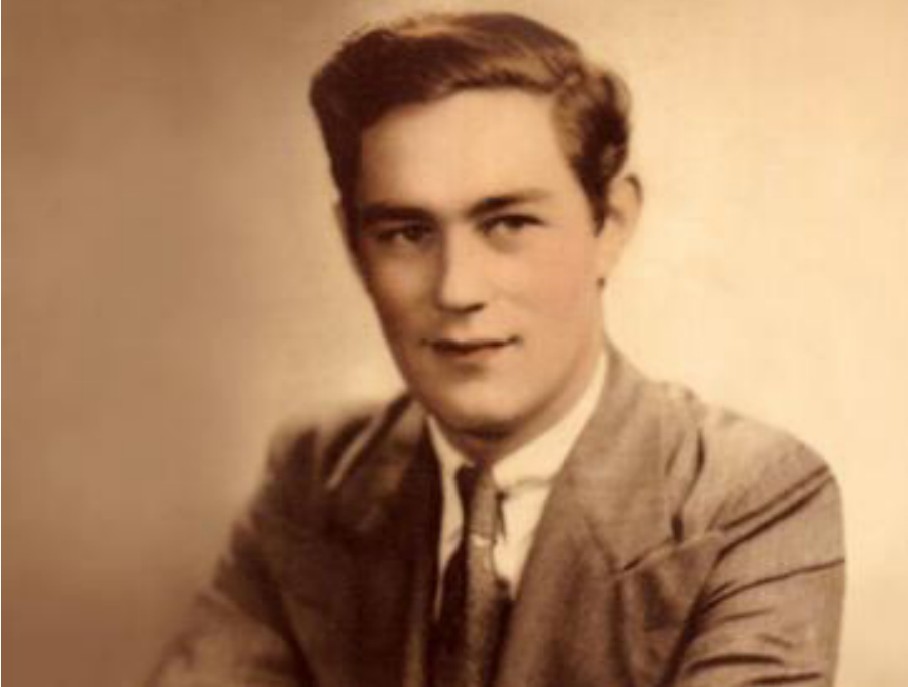
In his childhood, HM began having severe seizures, possibly the result of a head injury. In his teenage years, he started having tonic-clonic seizures, the most severe form of seizures that produces a loss of consciousness and convulsions (extreme muscle contraction or extension). In his early adulthood, he was having a tonic-clonic seizure monthly and several minor seizures daily, preventing him from working a normal job or living a normal life – despite taking a cocktail of anti-epileptic medications.
Neurosurgeon William Scoville proposed a “frankly experimental operation” to treat HM. It was known that most epilepsy originates in patches of neurons of the medial temporal lobe (MTL), and HM’s epilepsy was typical in this respect. Scoville suggested to surgically resect the MTL. In 1953, Scoville removed about 8 cm of the MTL bilaterally, including part of the amygdala, and notably the hippocampus, the seahorse-shaped structure of the brain.
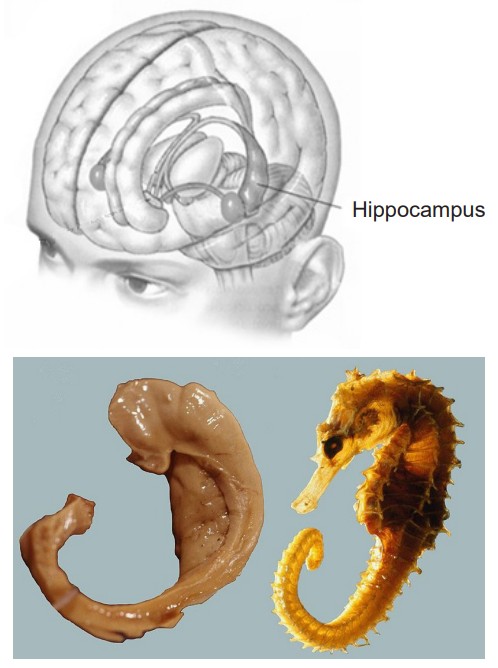
The surgery succeeded at its primary goal: HM’s seizures were less frequent and less severe. However, HM was left with a highly unusual and life-altering side effect: He was unable to create new discrete memories, a memory deficit called anterograde amnesia. For example, he could not remember what he had eaten for lunch just minutes after finishing the last bite. Despite being an avid fan of watching the news, HM couldn’t remember the names or the faces of different celebrities or public figures. It was as if he was permanently living in the present. (In contrast, retrograde amnesia affects the ability to successfully retrieve memory from one’s past.)
However, despite his pervasive memory deficits, HM did not display any deficits in intelligence. His language and speech were unaffected, and word recall was excellent, as he loved completing crossword puzzles and often did so successfully late in life, with only the occasional spelling errors. He could learn to acquire new skills, such as keeping a pen still on a moving circular platform, or a tapping task (these skills are different form of memory called procedural memory; see below). He was also capable of recalling things from his early childhood, such as geography facts he had learned in elementary school.
Types of memories
The fact that HM’s MTL surgery disrupted some types of memories (e.g., memory for facts) while others were still intact (e.g., motor skills) inspired neuropsychiatrists to try to define the different forms of memory. Much of the research was led by Dr. Brenda Milner, who carried out several behavioral tests on HM to figure out what types of memories are dependent on the intact MTL and which ones can function without MTL.
The most profound deficit was HM’s inability to create new declarative memories. Declarative memories, also called explicit memories, are the pieces of information that can be consciously declared or stated explicitly. Declarative memories are thought of as a “knowing what”. Declarative memories can be further subdivided into semantic memory and episodic memory.
Semantic memories are pieces of factual information. Some examples include:
1. “Jupiter is the largest planet of our solar system.”
2. “Rosalind Franklin discovered the double-helix structure of a DNA molecule.”
3. “The actor Keanu Reeves played the protagonist of the movie The Matrix.”
An episodic memory, sometimes also called an autobiographical memory, is the recollection of a discrete moment in a person’s life. It can be thought of as “mental-time travel” – what was it like when. The following memories are examples of episodic memories:
• “When I got home, I put my wallet and phone on the table.”
• “I ordered pizza last night.”
• “In 2019, I went to see my favorite musicians perform live.”
Several tests concluded that HM had lost his ability to create new semantic memories. In one such study, HM was asked to determine if a word was made up or real. He was shown words with very old origins, such as “shepherd” or “butcher.” On these words, he performed as well as the control group. When he was shown words that are made up, such as “phlage” or “thweise”, he likewise performed as well as the controls. However, when shown words that were added into the dictionary after his 1953-surgery, such as “granola” or “jacuzzi,” he scored about 50% correct – consistent with guessing at random, as if he never acquired the knowledge that these words have a meaning.
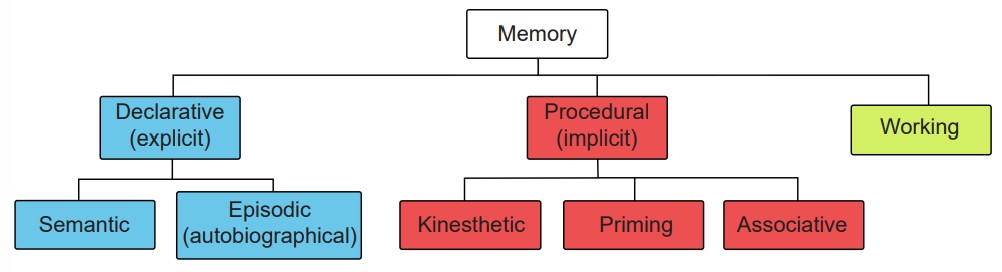
HM was also unable to create autobiographical memories. When asked to recall one of his birthday celebrations as an adult, he wouldn’t be able to give any significant details about the event. Instead, his answers were often vague and generic.
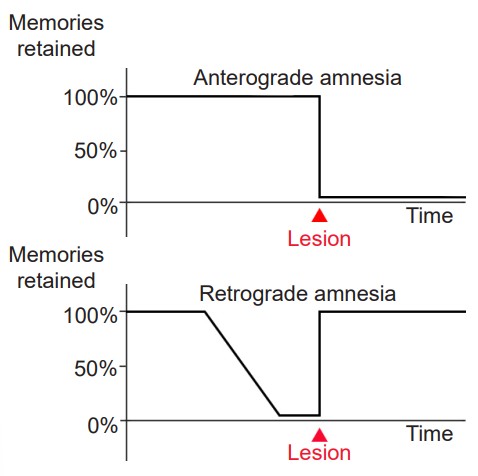
One interesting observation was that HM’s memory about details from his childhood were still intact. The inability to recall memories from the past, in this case, from before HM´s surgery, is called retrograde amnesia. Patient HM’s retrograde amnesia was temporally graded, meaning that the farther back you examine, the more complete his memories were. Many of his memories for the two years before his surgery were completely lost, but memories from his youth and teenage years were intact as much as healthy individuals (there is contention about this observation, because HM was taking several anti-epileptic drugs, which may have impacted memory formation.) From this observation, memory researchers concluded that the MTL functions as short-term storage site for memories, but after some years, those memories get relocated to other brain areas outside of the MTL. Currently, the scientific evidence suggests that memories are distributed across several networks of cortical and subcortical brain areas.
While HM lost the ability to create new declarative memories, he was still able to maintain a different class of memories, called procedural memories (or implicit memories). They are unconscious memories, and can’t be explicitly stated. These can be thought of as “knowing how”. Some examples of procedural memories include, for example, performance of a series of motor actions without conscious thought such as an experienced musician playing a simple scale (sometimes commonly called “muscle memory”, even though the muscles do not store any actual memory!), or a priming effect (such as when a person sees pictures of bananas, they are more likely to answer the fill-in-the-blank prompt “b _ _ _ _ _” with “banana”, whereas other people might guess “bubble” or “badger”).
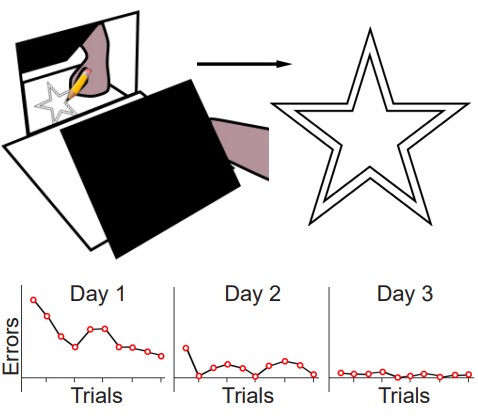
The original test of procedural memory conducted by Dr. Brenda Milner was called the mirror tracing task. In this test, the patient is told to draw a third star in between the two stars as quickly as possible without making any mistakes. The challenge is that the tracing is to be done while watching their hand and the star in their reflection in a mirror. Because of these unusual circumstances, completing this task is difficult. But over multiple days of practice, people become better at this mirror tracing task, completing it faster with fewer errors. Improvement on this task indicates that a person is learning or gaining some memory about how to better perform the task.
After practicing this mirror tracing task, HM was able to finish drawing the star about ten times faster than when he first began. He improved his performance within each day’s worth of training, and he also improved day-to-day. There is evidence that he maintained these skills up to one year later, despite not having regular training on this task. Surprisingly, each day Milner examined HM, she would need to reintroduce herself since he forgot who she was. She also had to re-explain what HM was supposed to do in the mirror tracing task. Hence, while HM was unable to form declarative memory about the experiment or the people involved, learning of the procedural memories and motor actions involved in this task remained intact.
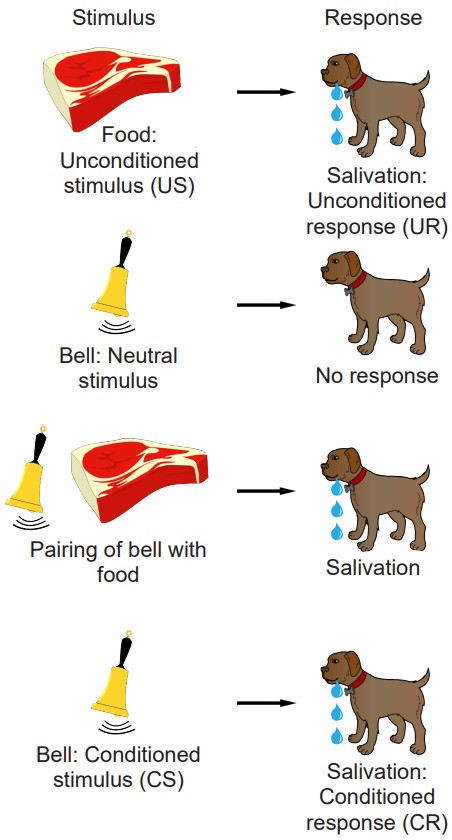
Another type of procedural memory is an associative memory. Associative memories are the types of information that we learn through traditional Pavlovian conditioning. For example, recall the classic Nobel prize-winning experiment in physiology conducted by Ivan Pavlov in the late 1800s. Normally, the presentation of dog food, an unconditioned stimulus (US), causes a dog to salivate, a naturally happening behavior, called the unconditioned response (UR). Dogs are not particularly interested in the sound of a whistle: this neutral stimulus will produce a minor response, such as a head turn and attentional shift towards the origin of the sound, but not much more than that. However, when this stimulus is repeatedly paired with the presentation of food, dogs quickly learn to associate that the whistle signals food. After multiple pairings, upon hearing the whistle, a conditioned stimulus (CS), the dogs begin to salivate, a conditioned response (CR), independent of any food being presented.
Separate from declarative or procedural memories, a different form of memory called working memory was tested in HM. Working memory involves processes of storing information temporarily while simultaneously manipulating those pieces of information. This type of memory can be thought of as a “short-term memory on overdrive.” Although HM struggled with working memory immediately after his surgery, several years later HM performed as well as age-matched control patients on these tasks.
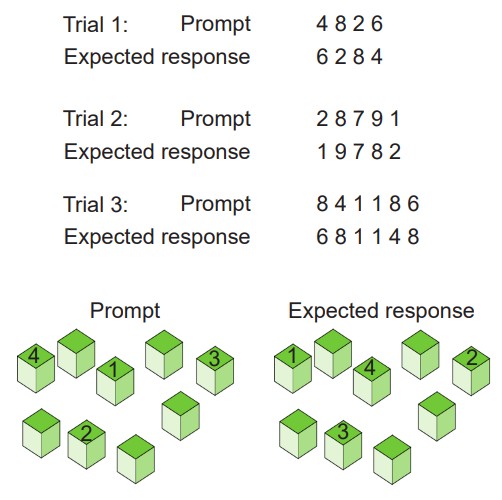
For example, a test of working memory is the digit span test, where a person is given a series of numbers to remember, then they are asked to repeat the numbers in reverse order. After successfully completing this task, a different series of numbers, this time one digit longer, is presented to the patient until they first start making errors in recall. A related task is called the Corsi block tapping test, where an experimenter sets up several blocks on a table. The experimenter then taps a series of blocks in a specific order, then the subject is asked to tap on the blocks in reverse order. As with the digit-span test, the experimenter then makes the series of blocks longer until they make mistakes in the tapping.
Patient HM died in 2008 at age 82 of respiratory failure. His name was Henry Molaison.
13.2 Neural Structures Involved in
The Hippocampus (HPC)
The hippocampus (HPC), meaning “seahorse” in Greek, was named based on its morphology. The HPC is located along the ventral and medial surface of the brain. The HPC is one of the critical structures of the limbic system, a series of subcortical brain structures that are involved in several different complex behaviors, such as emotions and memory. The limbic system is an evolutionarily ancient brain network.
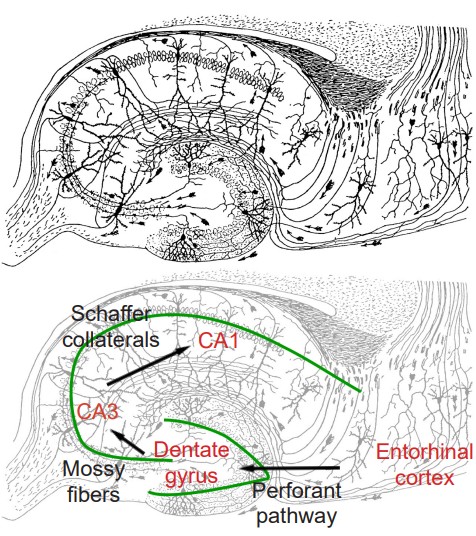
The synaptic connectivity of the hippocampus is very well characterized. Hippocampal synaptic connectivity was first described by Ramon y Cajal, and is made up of three main synaptic connections; sometimes called the trisynaptic circuit. First, the axonal outputs of layers 2 and 3 from the entorhinal cortex make up the inputs into the HPC. This white matter signaling tract is called the perforant pathway, and they synapse onto the granule cells of the dentate gyrus. These neurons send axons, called mossy fibers, to the pyramidal cells of the Cornu ammonis (CA) 3 region of the HPC. The axonal projections from here, called Schaffer collaterals, project into CA1, which are the neurons that make up the output of the hippocampus. These outputs project out to layer 5 and 6 of entorhinal cortex. While the three main neuronal projections are glutamatergic, the trisynaptic circuit is modulated by GABA, acetylcholine, norepinephrine, and serotonin.
The HPC is involved in spatial memories, memories involved in navigation of our surroundings and the creation of a mental map of our world. Spatial memories are developed when we enter a new building for the first time, and we search for a new classroom. We also use our spatial memory whenever we are walking around campus, making our way from one building to another, thinking about the streets you’d need to cross or the buildings you can cut through. While the volume of the hippocampus is not a reliable indicator of the strength of a healthy person’s spatial memory, injury to the hippocampus causes deficits in spatial memory.
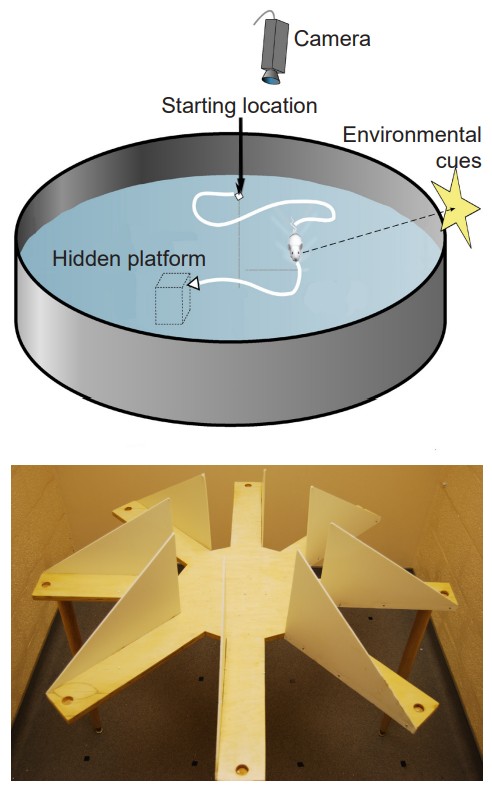
To test spatial memory behaviorally in non-human animals, one test that is regularly used in rodents is called the Morris water maze. In this test, a shallow pool is filled with an opaque liquid, making it difficult to see through. Hidden somewhere in this pool is a clear plexiglass platform, surrounding the pool are different environmental cues that can be seen from the surface of the water, such as different shapes or colors. The water is deep enough that when a rodent is put into the Morris water maze, they have to swim to stay afloat. The rodents swim around aimlessly until they find the platform, the time it takes for this to happen is recorded, and the trial ends. Over time, the animals learn that the platform is located near certain navigational cues, and on future trials, the animals spend more time near those cues, and the latency to find the platform decreases. When the HPC is surgically removed from rodents or inactivated, they perform poorly in the Morris water maze.
Another non-human behavioral test used to assess the capacity for learning navigational cues is the radial arm maze. In this test, a rodent is placed on a circular platform. Extending from this platform are eight or more “arms”, at the end of each is a small dish. In one of the dishes is a morsel of food (“rewarded arm”), while the other dishes contain nothing (“non-rewarded arms”). The maze is designed so that the food cannot be seen from the end of each arm, so the animal must return to the starting platform before exploring another arm. The number of entries into a non-rewarded arms is counted as an error. Over time, the animals make fewer errors as they learn which arm is rewarded and which ones are not. Alzheimer’s disease model organisms perform poorly on this task.
Based on the deficits seen in Patient HM and other experimental manipulations of the HPC, we conclude that the HPC is strongly implicated in the process of declarative memories and spatial navigation. Since some of HM’s memory functions were still intact, such as procedural memories and working memory, it is believed that these functions are independent of HPC function.
The Amygdala
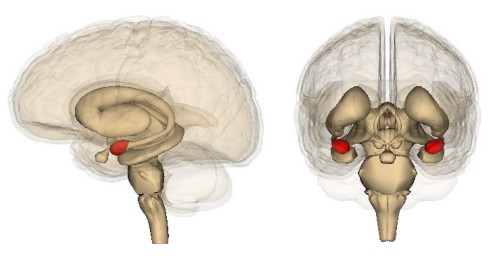
The amygdala is another limbic system structure found in the medial temporal lobe adjacent to the HPC. Amygdala comes from the Greek word meaning “almond,” which roughly describes its shape. While the amygdala is often spoken of as a single structure, it is more accurately divided into several subnuclei, each with different cell populations and functions. One broad division distinguishes the basolateral amygdala (BLA) versus the central nucleus of amygdala (CeA): The BLA contributes to both fear memories and reward processing, while the CeA contributes more to the physiological response in emotions as well the perception of emotion.
The amygdala is strongly involved with the formation and storage of emotional memories, memories or associations that have a strong emotional connection. Both positive and negative emotional states are represented here. For example, a whiff of grandmother’s cooking may cause you to reminisce back to a fun childhood summer. Alternatively, the smell of vomit may elicit the unpleasant emotions and nausea associated with a nasty food poisoning incident.
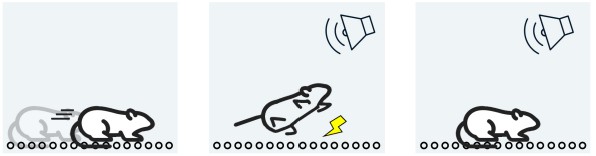
One non-human test of emotional memory is the foot-shock paradigm, a form of fear conditioning. This test involves putting a rodent into a chamber with floors made of metal rods, which are connected to an electric current generator. The metal rods can deliver a nonlethal but painful electric shock to the rodent’s foot. In this learning paradigm, a combination of sound and light cues is presented to the animal. Shortly after, the painful foot shock is delivered. If the animal learns that the cues are associated with the negative painful memory, they exhibit freezing after exposure to the cues. Amygdala lesions prevent the animal from freezing, while hippocampal lesions have no effect on this emotional learning. Changing cellular signaling in the amygdala alters the learning of fear conditioning. The foot-shock paradigm is often used as a non-human model of post-traumatic stress disorder.
Inferotemporal cortex (IT)
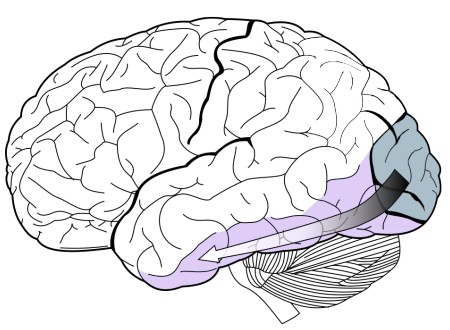
Structures of the inferotemporal cortex (IT) are part of the ventral stream of visual perception (chapter 7). The IT stores some components of visual memory. We use these functions when we see a classmate outside the classroom and recognize them from our Introduction to Neuroscience class, or when we see a parody of a famous painting and recognize the similarities to the original work. A simple behavioral task to assess visual memory would start by viewing a series of abstract shapes, and when a shape appears that you have already seen, you push on a button. The human capacity for visual memory is massive: After viewing 10,000 images for a few seconds apiece, people were able to identify a previously seen image successfully about 83% of the time.
A bit more specifically, one part of the IT is the fusiform gyrus, which has been previously described in the context of facial recognition. People with prosopagnosia, a visual perceptual disorder affecting the fusiform gyrus, can perceive the different parts of a person’s face, but have a difficult time putting the whole picture together and matching those features to a specific person. For facial recognition to be accurate, there must be some memory that allows for a person to match those facial features with someone they have seen before, which is a memory related process.
The parahippocampal place area (PPA), also found in IT, contributes to visual memories associated with locations and environmental scenes. Imaging studies have demonstrated that activity of the PPA increases specifically when people view place-related images, including scenic landscapes like mountains, man-made structures like campus buildings, or the interiors of rooms, both furnished and completely empty. To serve as control stimuli, viewing faces or objects does not increase the activity of the PPA.
Prefrontal Cortex (PFC)
As part of the frontal lobe ,the PFC is involved in high order decision making and personality. In the context of memory, neural circuits in PFC are important for short-term and working memory. Patients with injuries to their prefrontal cortex after stroke, tumors or aneurysm, performed worse on a variety of working memory tasks such as the digit span test. Additionally, people with frontotemporal dementia, a neurodegenerative disorder characterized by a degradation of the frontal lobe, often have difficulty with working memory.
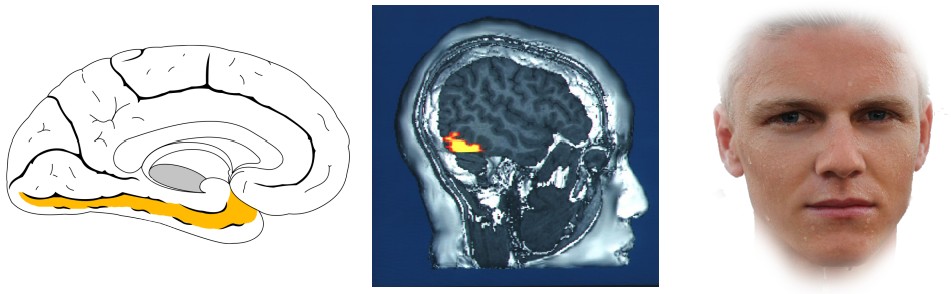
The PFC also has strong projections with the hippocampus, and these circuits are likely also involved in the formation of hippocampal-dependent memories.
Striatum
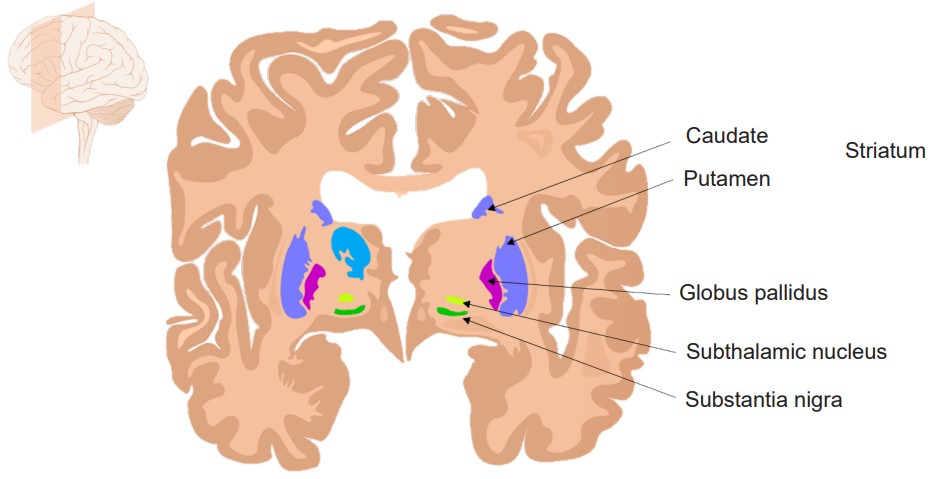
The striatum is a structure of the basal ganglia, a series of brain structures that contribute to behaviors such as motor activity (chapter 10) and procedural memories. The striatum likely holds memories involved in habits. Habitual behaviors help us preserve cognitive bandwidth, reducing the “mental energy” that is used during repetitive task performance. The downside of habits is that reliance on habitual responding can limit behavioral flexibility, and cause a person to act in a suboptimal manner, perhaps behaving in a way that led to a positive outcome in a previous set of circumstances without incorporating and evaluating the present circumstances.
Habitual action performance is likely related to a variety of neuropsychiatric disorders. Obsessive compulsive disorder (OCD), for example, is characterized by the presence of recurring, intrusive thoughts, which can lead to repetitive actions. Commonly observed is the thought that one’s hands are unclean, which leads to repeated handwashing.
A rodent behavioral test of habitual activity is the observation of self-grooming, a natural and healthy series of stereotyped actions that consists of licking the paws and moving them through the fur of the nose, caudally down the body. Mouse models of OCD show excessive self-grooming to the point where they pull their fur out and paw their skin to the point of injury.
Drug addiction is also a striatal disorder. Compulsive drug use is often associated with a series of habitual motor actions that happen before a person experiences the drug effect. For example, in tobacco use disorder, people will perform an orchestrated series of actions, including opening a pack of cigarettes, flicking the lighter, withdrawing the cigarette and taking a deep inhalation. Some of these behaviors are likely stored across striatal circuits (chapter 11).
Cerebellum
The cerebellum is the phylogenetically ancient structure found posterior and ventral to the cerebrum, and functions generally to help with motor functions (chapter 10). The cerebellum is involved in procedural memories, particularly the performance of motor abilities. Learning new motor skills likely requires changes in the circuit strength of cerebellar neurons.
This list of brain structures involved in memory is certainly not exclusive. For example, the orbitofrontal cortex plays a role in positive emotional memories, and sensory cortices are important for the memories related to the specific stimuli that are processed in those areas. A single memory could likely be stored in several brain areas, much like a mosaic.
Hypermnesia
Solomon Shereshevsky was one of a handful of rare, clinically documented cases of hypermnesia, the capacity to recall nearly any memory with perfect precision, even after several years. Remarkably, he could “easily remember any number of words and digits, equally easily he memorizes whole pages from books on any subject and in any language and for a quite long time at that. Shereshevsky can accurately quote anything he was told ten or twelve years ago.” He received recognition in 1968 when his psychologist A. R. Luria published a case study in “The Mind of a Mnemonist.”
Today, we would describe Shereshevsky as being autistic with strong multimodal synesthesia. Also of note, he had significant deficits in executive function, difficulty with recognizing faces, and could not interpret abstract ideas.
13.3 Cellular Mechanisms of Learning
In the late 1800s, around the time when Golgi and Ramon y Cajal were engaged in intense debate about the organization of the nervous system, many neuroscientists came to a strange observation: the weight of the brain increases dramatically over the first 10 years of life, but not much more after that. Even though we learn lots of new facts and make lots of new memories in adulthood, the brain itself doesn’t grow in size. So how is it possible to store new knowledge if the brain is not making many new neurons?
Most likely, new pieces of information are held in the connections between cells, not just in the cells themselves. If our estimate of 150 trillion synapses per adult brain is correct, then it is possible that we could store all the knowledge and memories that we collect over our lifetime through some combination of activity across certain connections.
The activity at the cellular level is believed to take place on at least three different levels enabling us to build, store, and retrieve memories.
1. Encoding refers to the ability for brain circuits to store some piece of information. In real life, you are presented with countless stimuli simultaneously. Imagine walking down a busy street, and think of the number of different sights, smells, and tactile stimuli you experience. Storing memories is an energetically costly process, and we are limited in the fact that all of our sensory inputs cannot possibly get encoded. Instead, evolution has preferred to encode stimuli that are most salient pieces of information, such as perceptual cues associated with predators. Alternatively, information that we pay strong attention to can get encoded more strongly, like when we repeat a phone number to ourselves until we have a chance to write it down. It is also easier to encode novel information that “builds” on previous bits of knowledge, or information that is closely related to other well-established information, which is why analogies are such an effective way to learn new facts.
2. The process that enables memory storing is called consolidation, which makes the memory more permanent. In 1949, an early neuropsychologist, Donald O. Hebb, offered an explanation for how changes in synapses could possibly lead to a phenomenon as complex as learning. His theory, published in his text The Organization of Behavior, can be summed up in the phrase:
“Cells that fire together, wire together.”
In Hebb’s framework, repeated activity at a synapse within a circuit of neurons acts as a reinforcer signal that strengthens this synapse for future communication, making the next incoming signal more robust. Hebb also implies the inverse is true: when cells do not fire together, they weaken their connection. Through fine tuning of synaptic connections, some strengthening and others weakening, a lifetime of memories can be stored across a wide distribution of neurons. After a memory has been created, the specific circuit of neurons that represent that piece of information is called a memory trace or an engram.
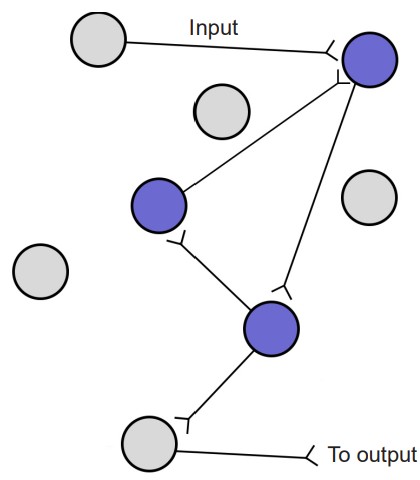
A cellular process called reverberation is thought to be the mechanism that allows for consolidation. Reverberation is the process by which networks of neurons fire repeatedly. Each time that circuit is activated, the strength of the network is increased, meaning that it becomes easier for that circuit to be activated in the future.
Throughout the process of consolidation, memory traces are thought to become more represented in the neocortex and less in the subcortical structures like hippocampus or amygdala. Recall Patient HM’s temporally graded retrograde amnesia, where he had lost declarative memories in the two years leading up to this surgery, and yet his memories from the long past were maintained. This finding suggests that some aspects of declarative memory consolidation depends at least partially on medial temporal lobe and HPC for a period of time, maybe up to two years, before those memories get stored in the cortex more permanently. However, we cannot conclude how long consolidation takes in healthy humans based on findings from HM, who had pervasive and frequent seizures, which might have negatively impacted memory consolidation before the surgery.
Consolidation seems to occur predominantly during sleep. Specifically, declarative memory is enhanced during non-REM sleep, while procedural memory is enhanced during REM sleep. Studies investigating sleep consolidation are often done by letting participants learn or perform behavioral tasks, after which they are deprived of a specific phase of sleep. To wake participants at the right moment, researchers commonly use EEG signatures, which are unique for different phases of sleep: sleep deprivation early in the night denies non-REM slow wave sleep, while late-sleep deprivation decreases time spent in REM sleep. Some speculate that the hallucinations we sometimes experience during dreaming are a consequence of consolidation processes, but it is inconclusive as to what role dreaming plays in memory formation.
3. Finally, for the stored memories to be recalled, a cellular process called retrieval happens which brings back the specific engram. Retrieval happens for both declarative and procedural memories.
The writing of memories can be differentiated from the retrieval of memories using specific priming-related behavioral tests. One example is a vocabulary recall test. Consider if you were given a list of 50 words to memorize, words that belong to a handful of different conceptual categories (such as cinnamon, pepper, or curry, which fall under the category “food flavors”). When asked to write down as many words as possible from the list using your memory, a test called free-recall, you may be able to successfully remember about a third of them. However, if you were prompted with the category titles, a related test called cued-recall, you would perform much better at retrieving those memories, possibly recalling up to 75% of the words. The fact that cued-recall scores are often higher than free-recall scores indicate that there is a distinction between the encoding / consolidation of memories and the retrieval of memories.
Retrieval is not a passive function. When an engram is retrieved, it is reconsolidated, which is an act similar to replaying the activity of the circuit. During this reconsolidation, it is possible that some aspects of the memory are emphasized, while others are lost. This is likely the main reason why we experience false memories, memories that are not true to reality – one reason why eyewitness testimonies are notoriously unreliable. We may imagine our good memories as better than they actually were, while simultaneously dampening the negative aspects of those memories. A dysregulation of this reconsolidation process could lead to the symptoms seen in post-traumatic stress disorder, where the negative emotional components of a particular memory are exaggerated rather than being blunted.
Special populations of neurons
An individual memory is likely distributed widely across several different parts of the brain. However, there are a few special populations of neurons mainly in the MTL that contribute to highly specific types of memories.
Place cells
Place cells are a special population of pyramidal cells of the hippocampus. These neurons increase their firing activity when the animal is in a particular location in an environment, indicating that they contribute strongly to location and navigational memory. There is no apparent topographical arrangement of these place cells, meaning that adjacent areas of an environment do not necessarily activate adjacent hippocampal place cells. The place cells, when firing at the right times, help the animal create a spatial map of their surroundings.
Grid cells
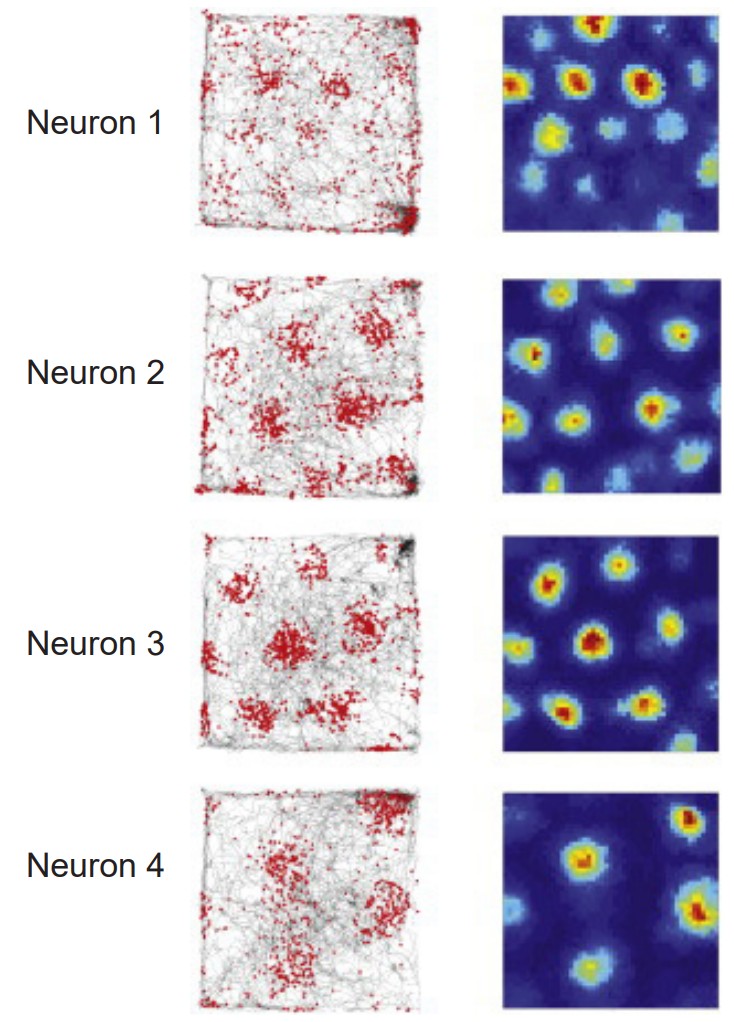
Grid cells are located in the entorhinal cortex, the main input structure to the HPC. Closely related to the place cells described above, grid cells increase their firing properties periodically when an animal is at an intersection of a “grid” in a wide-open, previously-explored environment. The grid itself is roughly hexagonal, and spans the whole environment an animal is in. The overlap of multiple grids gives the animal an idea of the surroundings. The scientific description of grid cells earned three scientists a Nobel Prize in Physiology or Medicine in 2014.
Jennifer Aniston neurons
The “Jennifer Aniston” neurons, also called concept cells, are a series of cortical neurons in the temporal lobe that increase their firing exclusively in response to highly-specific stimuli, such as the idea of Jennifer Aniston, Halle Berry, or the Tower of Pisa. These concept cells respond to much more than just pictures: for example, a Luke Skywalker neuron that responded to a picture of young Mark Hamill (the actor who played Luke Skywalker) will also respond to text that reads “LUKE SKYWALKER” and the sound of a person saying “Luke Skywalker”. Although this particular neuron probably won’t fire in response to pictures of athlete Manu Ginobili or actress Marilyn Monroe, the neuron might fire in response to pictures of Yoda or Darth Vader, indicating that the neuron may encode an even broader concept, such as “Star Wars characters” or “Jedi”, or is a part of a network that encodes concepts related to the Star Wars franchise.

13.4 Molecular Mechanisms of Learning
Zooming in beyond the level of anatomy, the substrates of learning can be found at the level of synapses. Synapses change in a phenomenon called plasticity. The word “plasticity” refers to a change in synaptic strength, which may be an increase or a decrease. This change may persist for minutes, hours, days, or in some cases, even a whole lifetime. When synaptic strength is increased and remains elevated, we call this long-term potentiation (LTP). A prolonged weakness of a synapse is called long-term depression (LTD). In our current limited understanding of plasticity, both phenomena are important for a healthy brain, and neither one is always good or always bad.
It is also important to clarify that both excitatory synapses and inhibitory synapses can be subject to either LTP or LTD.
Long-term potentiation
In 1973, Bliss and Lomo were the first to publish evidence of plasticity using electrophysiology. The experiment began at the hippocampal connections of an anesthetized rabbit. They put a stimulating electrode among the axons of the perforant pathway, at the entrance to the hippocampus. A second electrode, capable of detecting electrical charges in brain tissue, was placed among the cells of the dentate gyrus, the area where those axons release neurotransmitter. By stimulating the perforant pathway, Bliss and Lomo could record how neurons of the dentate gyrus respond. A single pulse caused the neurons to depolarize, a measurable observation called a field excitatory post-synaptic potential (fEPSP). The more neurons that depolarize, the larger the fEPSP would be.
Instead of simply giving a single electrical stimulation, however, Bliss and Lomo were interested in testing Hebb’s theory about plasticity. If “cells that fire together, wire together,” then perhaps they could experimentally drive those cells to fire in a pattern that would induce a rewiring of the connections, resulting in LTP. The duo delivered a very intense electrical stimulation, zapping the axons at 100 stimulations a second (100 Hz) for 3 seconds. This high frequency stimulation (HFS) led to an enhancement of the amplitude of the fEPSP in response to a single stimulus – this demonstrated that LTP was a measurable phenomenon. In a different experimental setup, this LTP was shown to persist up to one year later! In humans, we theorize that some synaptic connections may remain potentiated for our entire lifetime, however investigating this is in humans is ethically constrained.
Long-lasting changes in synaptic strength, such as the LTP that Bliss and Lomo demonstrated, are made possible through a series of molecular and cellular level changes. One form of LTP results from a change in the types of glutamatergic receptors. Of the three classes of ionotropic glutamate receptors, two are important for this form of LTP: the AMPA and the NMDA receptors. The AMPA receptors are the glutamate receptors that we generally imagine as contributing to excitation (more information in section 5.4). When a molecule of glutamate binds to the active site of this receptor, the ligandgated ion channel changes and allows cations, mostly Na+, to cross the cell membrane, leading to depolarization.
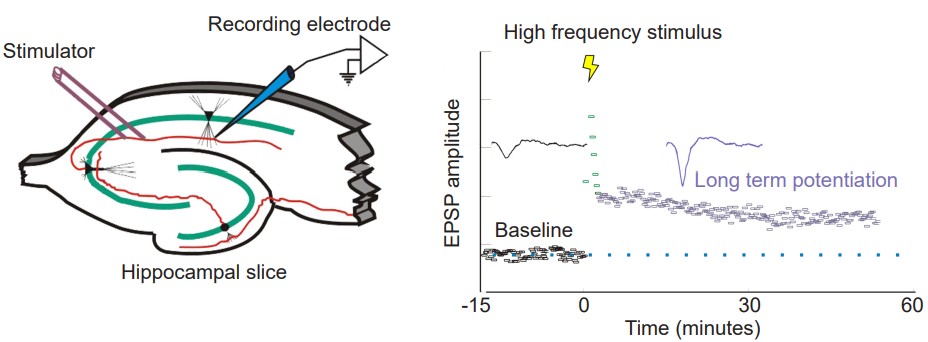
The NMDA receptors are somewhat more complex. NMDA receptors are like the ionotropic AMPA receptors because they are permeable to cations and therefore excitatory, but they have a few specific functional differences. For one, their molecular pore has space for a magnesium ion (Mg2+) to sit in the middle of the ion channel. Mg2+, like the other ions that we have discussed (chapter 4.2), responds similarly to the forces of the electrochemical gradient. Mg2+ is more highly concentrated outside the cell compared to the inside, and it has two positive charges, so these ions are drawn to the interior of the cell. But, the pore of the NMDA receptor is not large enough to allow the bulky Mg2+ ion to actually cross into or out of the cell membrane. Instead, it stays stuck inside the ion channel. Mg2+ physically takes up so much space that it occludes the movement of other ions across the cell membrane, basically blocking passage of ions through the NMDA receptor.
The other relevant feature of the NMDA receptor is that it is permeable to the Ca2+ ion. Increases in intracellular Ca2+ postsynaptically is the crucial trigger that leads to the cellular expression of LTP. Ca2+ ions activate an enzyme called calcium / calmodulin-dependent protein kinase II (CaMKII). CaMKII itself has many molecular targets. As a kinase, it’s main molecular action is to phosphorylate proteins. When CaMKII becomes activated, it phosphorylates amino acid residues on the AMPA receptor, which enhances their current passing properties, thereby increasing their response with glutamate present. Secondly, CaMKII also contributes to cellular mechanisms which result in increased trafficking of AMPA receptors to the cell surface. Thirdly, CaMKII interacts with the transcription factor cAMP response element-binding protein (CREB), which can then move into the nucleus and instruct the nucleus to synthesize more of the mRNA that leads to increased synthesis of the AMPA receptors. Taken together, increases in intracellular Ca2+ postsynaptically leads to an enhancement of a signal that persists over the time course of hours: The definition of LTP.
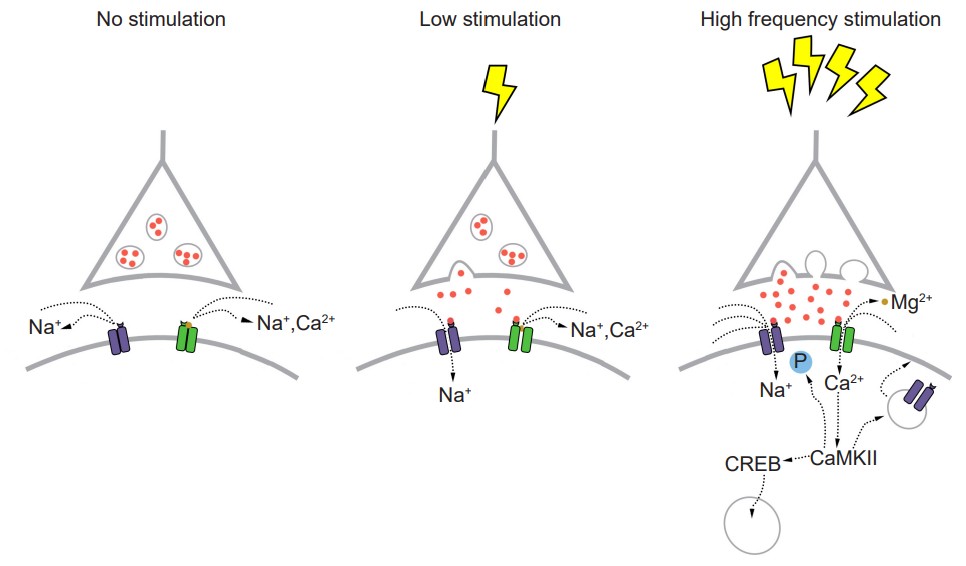
But, these NMDA receptors are not activated by glutamate alone. Because of the molecular properties of the NMDA receptors, they need two conditions to be fulfilled before these receptors get activated and Ca2+ moves into the cell membrane:
1. A ligand like glutamate must activate the receptor. As with other receptors, there is no activation in the absence of an agonist.
2. The postsynaptic cell must also be depolarized. When the cell is at positive potentials, the electrical gradient causes the bulky Mg2+ ion that is stuck in the pore to be repelled by the cell’s interior, which then frees the ion channel for movement of cations across the cell membrane.
Because both conditions must be met before Ca2+ can trigger plasticity through CaMKII activation, the NMDA receptor can be described as a coincidence detector. These properties can help explain why LTP was only observed after Bliss and Lomo activated the hippocampal slices robustly with their high frequency stimulus paradigm. Strong activation depolarized the axonal fibers, which caused a significant amount of glutamate to be released, activating many of the postsynaptic AMPA receptors. This strong activation caused the postsynaptic neurons to depolarize, which expels the Mg2+ ion out of the NMDA receptor. At this stage, both conditions are fulfilled, and Ca2+ enters into the postsynaptic cell, which activates CaMKII, triggering LTP.
Some glutamatergic connections between neurons contain only NMDA receptors but no AMPA receptors. Because these postsynaptic cells do not depolarize in response to glutamate release, and no current passes through the NMDA receptor due to the Mg2+ block, these synapses do not change their activity even with glutamate release. These synapses are called silent synapses. As we grow, the number of silent synapses decreases, another aspect of brain development.
Long-term depression
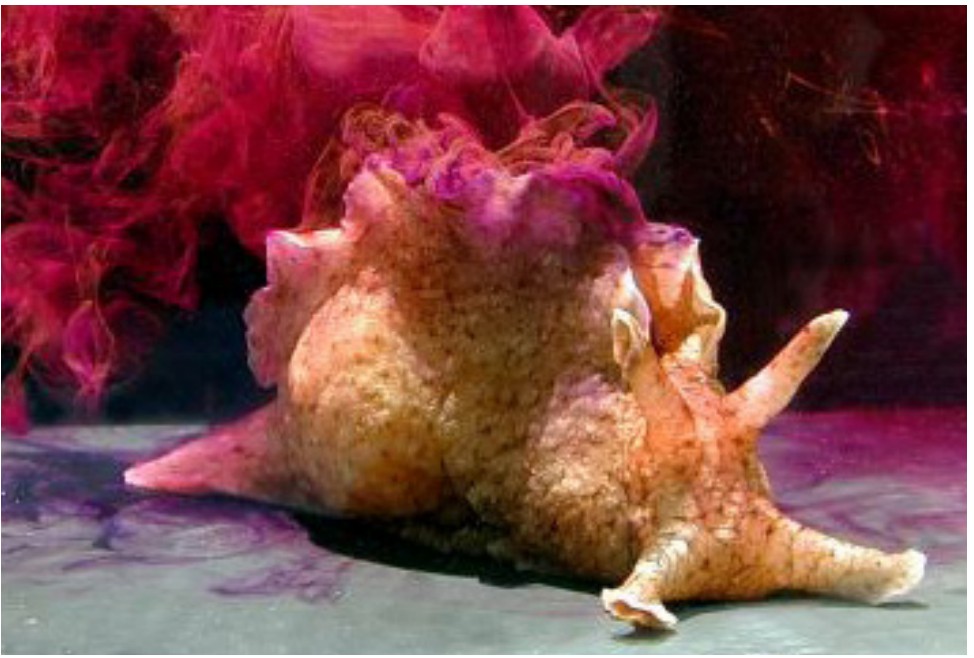
Around the same time LTP was being characterized in the rabbit hippocampus, its cellular opposite, long-term depression (LTD), was also being demonstrated in a different experimental preparation. Through the 60s, psychiatrist Eric Kandel and his colleagues worked with the marine mollusk Aplysia californica. With a nervous system of only 20,000 cells, Aplysia is orders of magnitude simpler than the other model organisms used at the time. Additionally, some Aplysia neurons are huge, up to a millimeter in diameter, which took away the need for highly precise equipment.
Aplysia also has a relatively simple anatomy. It breathes using a half-circle of delicate tissue called the gill, which is guarded by the mantle shelf. They also have an organ called the siphon, a small tube that is used for moving water through the animal. Kandel and his colleagues began their exploration of memory by studying the gill-withdrawal reflex, a defensive motor response behavior. When a stimulus, such as a hungry predator (or an experimenter’s paintbrush), grazed the siphon, the Aplysia would reflexively withdraw their gill, as if to protect this vital organ by shrinking away from the threat. However, after repeated brush strokes to the siphon, the sea slugs figured out that the stimulus was completely innocuous, and decreased the strength of gill withdrawal. Kandel and team suggested that this change in behavior was a form of learning.
Kandel discovered evidence of habituation, the suppression of a normal reflex behavior that is dependent on LTD. To further explore the cellular and molecular level changes behind this LTD, they conducted electrophysiological experiments on Aplysia. The gill-withdrawal reflex circuit relies heavily on two different populations of neurons: the sensory neurons that receive somatosensory information from the skin of the siphon, and the motor neurons that control the muscles of the gill. By using two different tiny glass pipettes, they could impale these neurons, inducing action potential firing in the sensory neuron, and observe changes in membrane potential of the motor neurons (a depolarization of the membrane of a single neuron is called an excitatory postsynaptic potential, or EPSP). When the sensory neuron was activated, they observed an EPSP in response, since an action potential caused release of glutamate that activates post-synaptic receptors on the motor neuron. However, after the reflex had been habituated, the same sensory neuron activation caused a much smaller EPSP in the motor neuron.
The group went about seeing if they could modify this habituated response, curious if a stored memory can be modified by stimuli from the outside world. When they paired the mild siphon touch with a painful electric shock to the tail, the Aplysia began responding with a strong motor reaction, withdrawing the gill very intensely, indicating that the inhibited response disappeared. They called this observation sensitization. In electrophysiological studies, they observed that the EPSP at the motor neuron was much larger following the tail shock.
On a molecular level, presentation of sensitization is downstream of the action of a third population of neurons, interneurons that synapse onto the motor neurons. The noxious stimulus triggers these interneurons to release the neurotransmitter serotonin, which activates the excitatory signaling molecule cAMP found at the terminals of the motor neurons, thus increasing release probability and strengthening the gill withdrawal reflex.
Other forms of plasticity
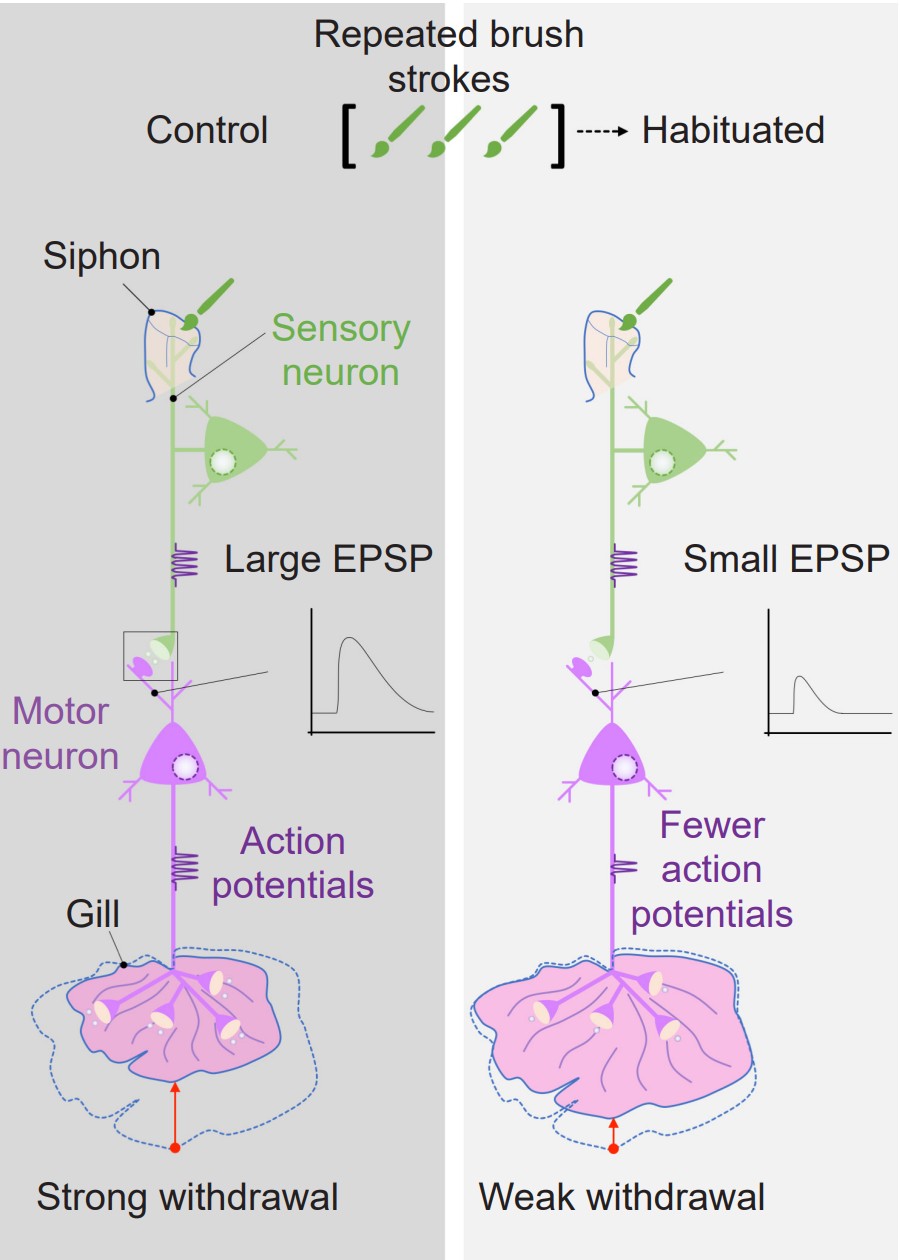
There are many possible mechanisms that can lead to plasticity. Some activity patterns may induce a short-term plasticity that does not last on the scale of hours or years, but rather on the order of minutes or seconds.
One important form of plasticity is dependent on the activity of the endocannabinoid signaling pathway. During robust post-synaptic depolarization, enzymes such as phospholipase C or DAG lipase create signaling molecules called endocannabinoids (eCBs), which are chemically similar to the primary psychoactive ingredient of Cannabis (chapter 11). These eCBs are neurotransmitters that act in a retrograde manner, meaning they are produced at dendrites and signal to the axons. Expressed at some populations of axons are inhibitory Gi/o coupled receptors that respond to the presence of eCBs, called cannabinoid receptors (CB1 is the main form that exists in the brain). These receptors inhibit cAMP signaling, which decreases release probability. This specific mechanism of plasticity is called endocannabinoid-mediated LTD.
Another atypical neurotransmitter, the gas nitric oxide (NO), also contributes to a similar form of LTD. The enzyme nNOS synthesizes NO, which diffuses across cell membranes and can activate an intracellular receptor called soluble guanylate cyclase (sGC). SGC changes the activity of the signaling molecule cGMP, which can either lead to enhancement or depression of synaptic strength.
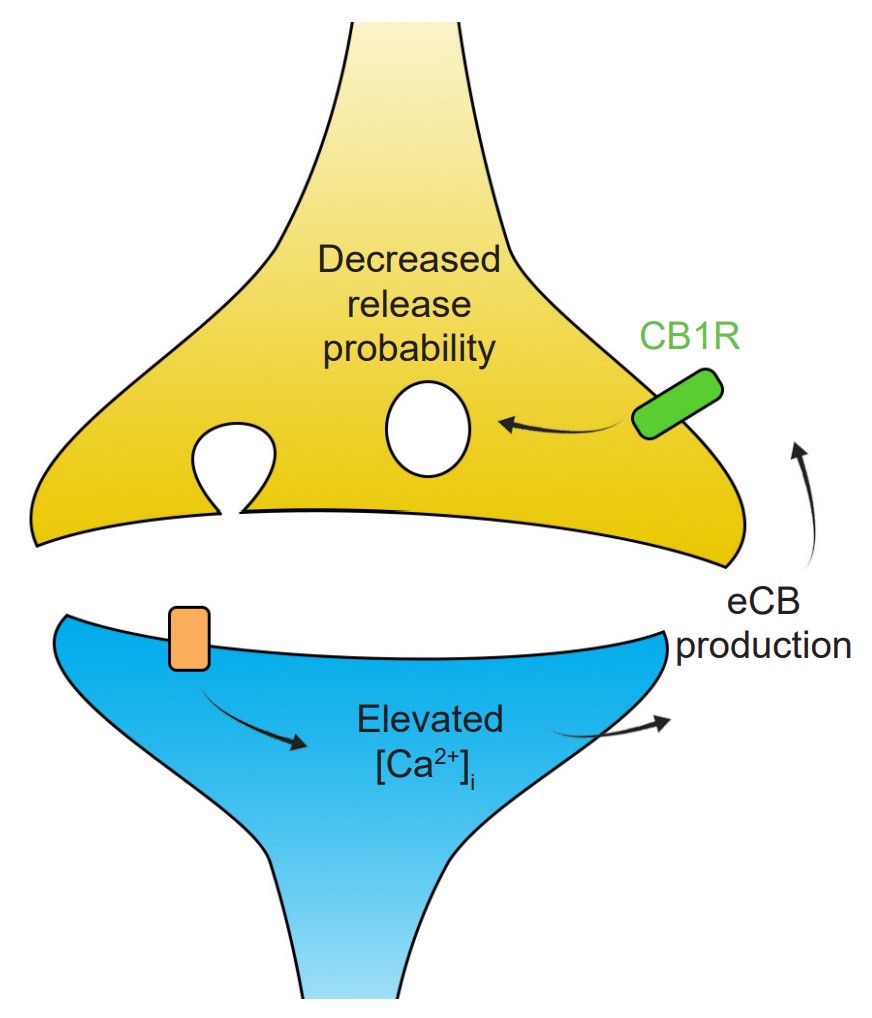
There are also two directionally-opposite forms of plasticity that function at the millisecond timescale, called paired-pulse facilitation (PPF) and paired-pulse depression (PPD). These forms of plasticity can be observed by stimulating a presynaptic area while recording simultaneously from the post-synaptic site. However, instead of delivering a single depolarizing pulse presynaptically, these forms of plasticity can only be observed by giving two pulses right after each other, usually separated in time by 10 milliseconds up to a second. In response to these depolarizations, you can also observe two EPSPs. Depending on the time between the pulses (called the interevent interval, or IEI) you may see that the second pulse is a different amplitude than the first. Dividing the amplitude of the second EPSP by the amplitude of the first EPSP gives us a value called the paired pulse ratio (PPR). If the amplitude of the second pulse is increased relative to the first pulse (PPR > 1), this is described as a PPF, whereas if the amplitude in response to the second pulse is smaller than the first (PPR < 1), this is PPD. Both of these plasticity phenomena can be observed at the same synapse by varying the duration of the IEI.
By understanding the molecular mechanisms of synaptic release, we can explain how PPF and PPD can occur at the same synapse. Recall that increased Ca2+ concentration is an important pre-synaptic intracellular signal that allows for vesicular fusion and neurotransmitter release (see section 5.2 for more details). Generally, pre-synaptic Ca2+ enters through voltage-gated calcium channels (VGCCs) that open during depolarization. Delivering a single pulse causes some Ca2+ entry, but giving two pulses, one right after another, allows for more robust activation of the VGCCs thus increasing the intracellular Ca2+ greatly, leading to more neurotransmitter release.
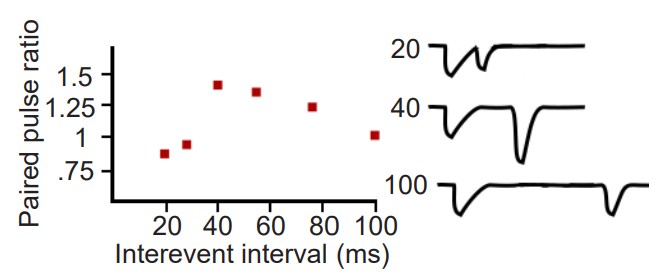
PPD can also be accounted for by looking at the localization of vesicles in the axon terminal. The readily-releasable pool are the vesicles that are close to the inside of the cell membrane. During a depolarization of the terminal, these vesicles are the first ones to fuse. However, the readily-releasable pool of vesicles can get depleted. On the second pulse, there may be a small number of vesicles remaining because vesicles need to be refilled before they can be released, which makes the amplitude of the second pulse small compared to the first. This is an explanation for paired-pulse depression.
13.5 Disorders of memory
Alzheimer’s disease
In 1907, Dr. Alois Alzheimer described one of his patients, a 50-year old woman named Auguste Deter, whose symptoms included profound cognitive impairment, memory deficits, and delusions. After Deter’s death, post-mortem analysis of her brain revealed anomalies: degenerating neurons that contained atypical tangles and deposits scattered between cells. These reports were the first of a disease we now call Alzheimer’s disease (AD). It is an irreversible, slowly progressing neurodegenerative condition that leads to deficits in thinking, behavior, and memory loss. Specifically, declarative memory is the first form of memory loss observed in AD patients. As the disease progresses, procedural memory loss becomes more apparent.
AD is a devastating disease that accounts for 60-80% of all cases of dementia and is the sixth leading cause of death in the United States. As for prevalence, approximately 10% of people Cortex Cortical atrophy Ventricular enlargement Hippocampus Entorhinal cortex Hippocampal shrinkage older than 65 and nearly a third older of people older than 85 has AD.
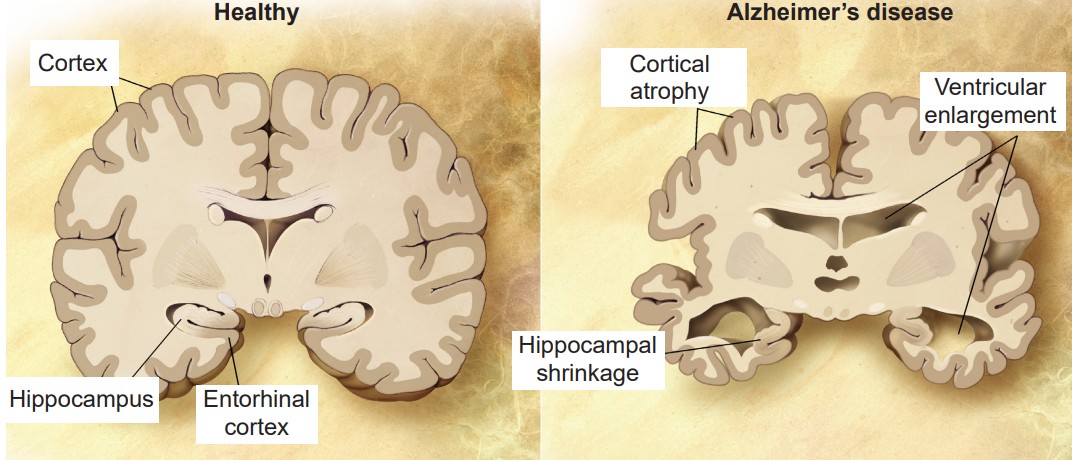
AD is divided into two categories, familial and sporadic. Familial AD is diagnosed when a person is in their 50s or 60s, and is strongly influenced by genetic risk factors. This form of AD only makes up about 10% of cases. Sporadic AD is by far more common than familial AD, and is believed to be caused by a combination of old age and environmental factors in addition to genetic risk factors.
Several genetic risk factors are linked to AD risk. Apolipoprotein epsilon4 (ApoE4) is the greatest genetic risk factor identified, where individuals who are homozygous for the e4 polymorphism have a 12-times higher risk of developing AD than people with the more common e3 variant. Additionally, mutations in genes like amyloid-precursor protein (APP), presenilin-1 (PSEN1) presenilin-2 (PSEN2), and triggering receptors expressed on myeloid cells 2 (TREM2) are all associated with higher risk of developing AD.
To this day, only post-mortem analysis can conclusively diagnose someone with AD. As expected, this raises many issues in terms of treating these patients. Currently, physicians diagnose a patient with AD based on the symptoms they presented with. However, new techniques to make more accurate diagnoses are emerging, including identifying the presence of biomarkers from blood samples, certain functional characteristics as observed in PET or fMRI imaging, and possibly even through eye exams!
An early hypothesis proposed to explain the neuronal loss and memory deficits observed in AD is centered around changes in the signaling of acetylcholine. Early post-mortem analyses discovered that people with AD have profound atrophy of the basal forebrain, an area which contains a large density of cholinergic neurons. The cholinergic hypothesis suggests that it is this loss of cholinergic neurons and the loss of acetylcholine signaling that is the main pathological driver of AD. The theory is supported by the idea that acetylcholine plays an important role in learning and memory. Three of the four FDA approved drugs for AD are acetylcholinesterase (AChE) inhibitors, drugs that act to increase levels of acetylcholine. Unfortunately, these compounds show only short-term benefits for cognitive function, and these therapeutic effects subside over time.
Pathologically, AD is characterized by the extracellular accumulation of amyloidbeta plaques (Aβ) and intracellular hyperphosphorylated neurofibrillary tautangles (NFT).
The amyloid cascade hypothesis, originally proposed in 1992, has become the leading theory in the field describing how AD develops. The hypothesis suggests that the main driving factor of AD is the deposition of Aβ in the brain, and this in turn leads to neurodegeneration via cell death, abnormal protein buildup, and neuroinflammation. Aβ is produced from the cleavage of amyloid-precursor protein (APP), an integral membrane protein expressed by neurons. A class of secretase enzymes are responsible for the degradation of APP. When the alpha and gamma secretases degrade APP, the resulting Aβ protein is likely to clump together in unpredictable ways, leading to the formation of Aβ plaques (pronounced “A beta”). These plaques can cause neuronal death and lead to the cognitive deficits observed in AD patients. Two major genetic risk factors for AD, presenilin 1 and 2, are mutations of the gamma secretase that leads to increased APP cleavage.
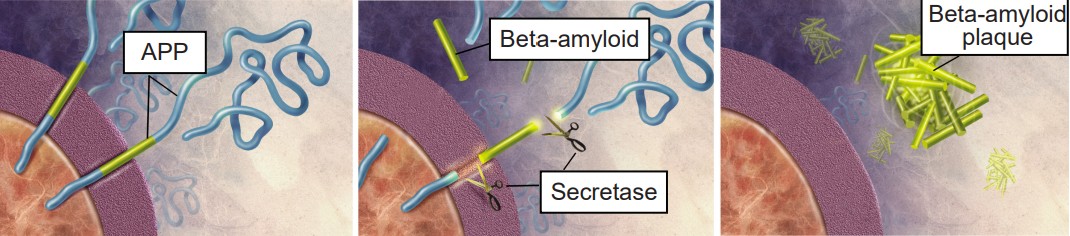
Therapies are being developed to target and control the Aβ protein to mitigate AD symptoms. Recently an antibody-based drug has been approved by the FDA. This groundbreaking drug is unique from those traditionally prescribed because it directly targets Aβ.
However, the amyloid-cascade hypothesis does not tell the whole story. For one, there are patients who carry a heavy Aβ load but present no clinical symptoms of AD. More so, in mouse models with APP mutations, they develop significant Aβ plaques, but have no accumulation of tau (see below) and no significant neurodegeneration. Additionally, there have been several drugs targeting Aβ that have failed in human trials.
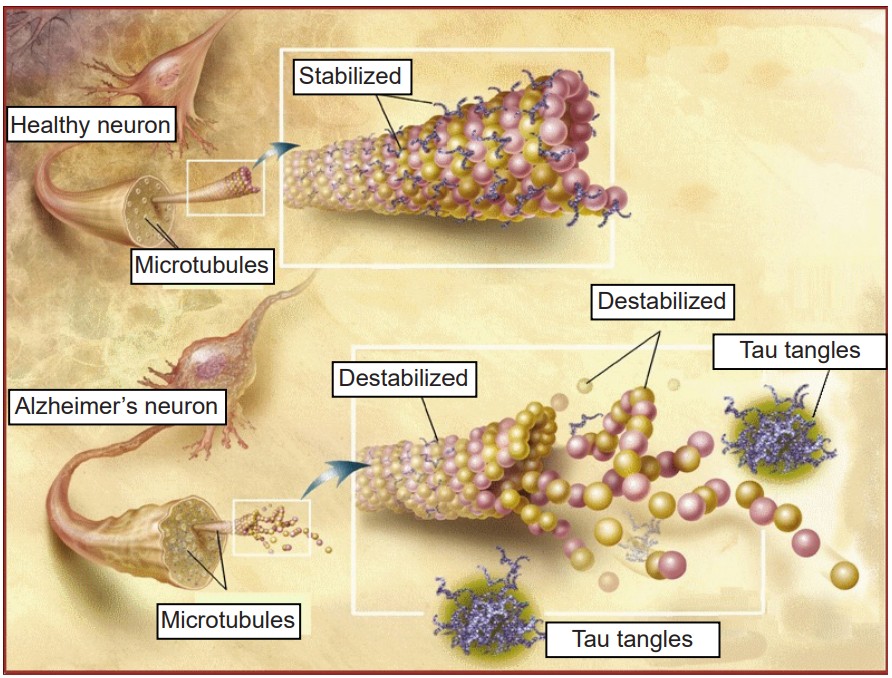
While these amyloid plaques seem to be the primary driving factor of AD, hyperphosphorylated tau and neurofibrillary tangles (NFTs) are also potential culprits in disease progression. NFTs are an intracellular pathological marker of AD and correlate strongly with cognitive deficits in AD. Tau protein is the major microtubule-associate protein (MAP) of mature neurons, and helps to function to maintain cellular morphology. Excess phosphorylation of this protein causes tau to accumulate inside the cell, leading to neuronal dysfunction and cell death. The presence of Aβ increases the levels of the tau, and vice versa, adding to the complexity and difficulty of treating AD. Tau pathology is also observed in other neurodegenerative diseases such as frontotemporal lobe dementia and Parkinson’s disease.
Although most discussion of AD revolves around plaques and tangles, it is well known that a cacophony of pathological markers are also seen in AD, including neuroinflammation, oxidative stress, blood-brain barrier dysfunction, heavy metal dysregulation, mitochondrial impairment, and many more.
Korsakoff’s syndrome
Korsakoff’s syndrome is a disorder resulting from a severe deficiency of thiamine, an essential vitamin that functions in metabolic processes. Dietary thiamine is found in whole grains, legumes such as beans and peas, as well as some meats and fishes. Healthy people with a well-balanced diet get sufficient thiamine, however gastrointestinal illnesses can cause an inability to absorb thiamine properly. Chronic alcohol misuse also impairs the body’s ability to take up thiamine, and is often the cause of Korsakoff’s syndrome.
People with Korsakoff’s syndrome experience both retrograde and anterograde amnesia, as well as severely impaired short-term memory. The patients may experience a very strange behavior called confabulation, which is the fabrication of false memories ranging from subtle to wildly fantastical. People who confabulate do not consciously recognize that their statements are untrue, and are not intentionally trying to deceive others, which is why it is sometimes called “honest lying”. Generally, confabulation only happens as a person is trying to recall recent autobiographical memories, while their semantic and procedural memories are less susceptible to confabulation. Scientists suggest that people confabulate as a compensatory mechanism to make up for their retrograde amnesia.
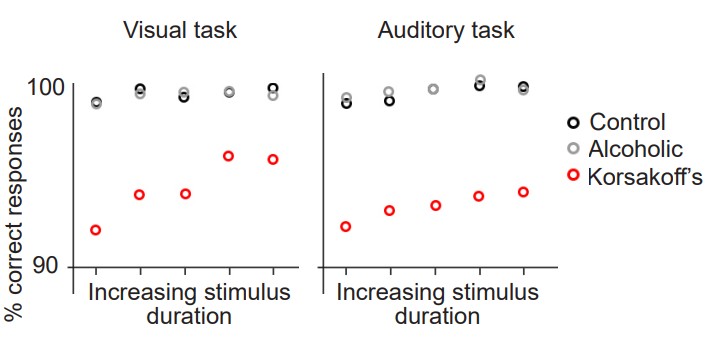
Destruction of both neurons and glia are seen in the brains of people with Korsakoff’s syndrome. As a result of this cell loss, there is often shrinkage of the cortex, thalamus, the hippocampus, cerebellum, and the mammillary bodies, paired structures located at the ventral surface of the brain close to the brain stem, themselves part of the limbic system.
Korsakoff’s syndrome can be treated by giving thiamine supplements and eliminating alcohol consumption. If treated within days after the onset of brain damage, people are expected to make a complete recovery. However, one of the major challenges is making a proper diagnosis, since the symptoms of Korsakoff’s syndrome present similarly to other disorders.
Traumatic brain injury
A sudden blow to the head is likely to produce a traumatic brain injury (TBI). TBIs are the most common form of brain damage, with about 2.88 million Americans in 2014 with a TBI-related emergency department visit, hospitalization, or death. People are exposed to these injuries regularly, such as in automobile accidents, falls, high-contact sports, occupational hazards such as in construction or military deployment, among many others. A concussion is a mild form of a TBI. Some of the common symptoms that people experience shortly after experiencing a TBI include double-vision, headache, dizziness, nausea and vomiting, and loss of consciousness, which are common symptoms in concussions as well.
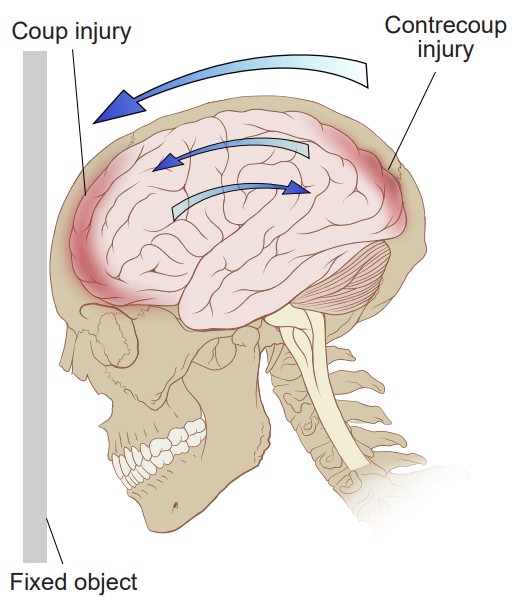
Usually in a traumatic brain injury, there are two simultaneous insults: the coup, which is the injury when the brain hits the inside of the skull closest to the external force. Shortly after, the contrecoup happens, which is the injury when the brain recoils backwards and hits the interior surface of the skull opposite of the cause of the insult. Following these injuries, there is a widespread inflammatory response that changes cellular activity in numerous complex ways, such as microglial activation, axonal stretching, and elevated levels of immune signaling molecules.
People with repeat head injuries may also experience some memory loss. It is suggested that the damage to the brain creates an abnormal cellular environment that interferes with the reverberation processes that are normally important for solidifying memories. Additionally, TBI decreases hippocampal neurogenesis, which is likely to impair the formation of declarative memories.
Savant syndrome
Savants are people with “islands of genius,” knowledge in extreme specializations, such as ability to calculate large prime numbers, identify the specific day of the week for any date, or recall sports trivia. Many savants have a passion for creative endeavors, such as in the visual arts or musical performance. About 20% of savants demonstrate extraordinary memory. The prevalence of savants in the general population is exceedingly low, but about 1 in 10 autistic people demonstrate some expression of savant skills.
While most savants are born with these skills, some acquire them as a result of a head injury. Orlando Serrell, after losing consciousness in a baseball accident, developed a complete autobiographical memory, able to remember where he was and exactly what he was doing on any given date after his injury. Following a diving accident, Derek Amato developed prodigious musical talents despite never having taken lessons, improvising on the piano as if playing through procedural memory.
One of the biggest difficulties is that TBI is very different from case to case, making development of new therapeutic strategies challenging. Currently, the best treatment for TBI is rest. There is still debate about the types of medications that can help best improve outcomes, psychostimulants and antidepressants being the most common classes of drugs used in TBI
Image Credits
13.1 https://en.wikipedia.org/wiki/File:Henry_Gustav_1.jpg
13.2 https://commons.wikimedia.org/wiki/File:Hippolobes.gif
https://commons.wikimedia.org/wiki/File:Hippocampus_and_seahorse_cropped.JPG
13.5 https://pixabay.com/vectors/pencil-sharp-school-supplies-153561/
13.6 https://commons.wikimedia.org/wiki/File:Classical_Conditioning_Diagram.png modified by Austin Lim
13.8 https://commons.wikimedia.org/wiki/File:CajalHippocampus.jpeg modified by Austin Lim
13.9 https://commons.wikimedia.org/wiki/File:MorrisWaterMaze.svg modified by Austin Lim
https://commons.wikimedia.org/wiki/File:Simple_Radial_Maze.JPG
13.10 https://commons.wikimedia.org/wiki/File:Amygdala.png
13.11 https://commons.wikimedia.org/wiki/File:202003_Model_animal_mouse_mono.svg
https://pixabay.com/vectors/sound-audio-music-icon-studio-2935370/
13.12 https://commons.wikimedia.org/wiki/File:Ventral-dorsal_streams.svg modified by Austin Lim
13.13 https://commons.wikimedia.org/wiki/File:Gray727_fusiform_gyrus.png modified by Austin Lim
https://commons.wikimedia.org/wiki/File:Fusiform_face_area_face_recognition.jpg
https://pixabay.com/photos/man-water-wet-male-face-swimming-984504/ modified by Austin Lim
13.14 https://commons.wikimedia.org/wiki/File:The_structures_of_the_basal_ganglia.png
13.16 https://commons.wikimedia.org/wiki/File:Examples_of_Grid_Cells_with_Different_Grid_Spacing_and_Field_Size.jpg
13.17 https://commons.wikimedia.org/wiki/File:Mark_Hamill_(right)_and_me_(left)_(210269249).jpg
https://commons.wikimedia.org/wiki/File:Mark_Hamill_(1980).jpg https://commons.wikimedia.org/wiki/File:Star_Wars_
characters_at_Madame_Tussaud.jpg
https://commons.wikimedia.org/wiki/File:Retrato_del_Maestro_Yoda.jpg https://commons.wikimedia.org/wiki/
File:Leonardo_DiCaprio_2014.jpg https://commons.wikimedia.org/wiki/File:Keanu_Reeves_2014.jpg
13.18 https://commons.wikimedia.org/wiki/File:LTP_exemplar.jpg modified by Austin Lim
13.19 https://commons.wikimedia.org/wiki/File:Potential_mechanisms_of_LTP_in_spinal_dorsal_horn_in_vivo.jpg
modified by Austin Lim
13.20 https://commons.wikimedia.org/wiki/File:Aplysia_californica.jpg
13.21 https://commons.wikimedia.org/wiki/File:Habituation.png
13.24 https://commons.wikimedia.org/wiki/File:Alzheimer%27s_disease_brain_comparison.jpg
13.25 https://commons.wikimedia.org/wiki/File:Amyloid-plaque_formation-big.jpg modified by Austin Lim
13.26 https://commons.wikimedia.org/wiki/File:TauProtein.jpg modified by Austin Lim
13.27 https://upload.wikimedia.org/wikipedia/commons/a/a8/Alcohol-induced_brain_damage_%28IA_
alcoholinducedbr00hunt%29.pdf modified by Austin Lim
13.28 https://commons.wikimedia.org/wiki/File:Contrecoup.svg
One of the most influential case studies in the neuroscience of memory. HM experienced life-long anterograde amnesia after the removal of the hippocampus in his brain
the most severe form of seizures that produces a loss of consciousness and convulsions
patches of neurons where most epilepsy originates
helps to remember important facts
inability to create new discrete memories
inability to successfully retrieve memory from one’s past
the learning of motor skills
the pieces of information that can be consciously declared or stated explicitly
another term for declarative memories
pieces of factual information
the recollection of a discrete moment in a person’s life
another term for episodic memory
in someone with retrograde amnesia- the farther back you examine, the more complete memories are
another term for procedural memories
The original test of procedural memory
the types of information that we learn through traditional Pavlovian conditioning
stimulus leading to an unconditioned response
a naturally happening behavior in response to a stimulus
a previously neutral stimulus that now leads to a conditioned response
response to the conditioned stimulus; usually the same as the unconditioned response
involves processes of storing information temporarily while simultaneously manipulating those pieces of information
a common test of working memory
a common test of working memory
a series of subcortical brain structures that are involved in several different complex behaviors, such as emotions and memory
the three main synaptic connections that make up the hippocampus
makes up the inputs to the hippocampus
white matter signaling tract of the hippocampus
contains the perforant pathways on its granule cells
axons sent to the pyramidal cells of the CA3 region of the hippocampus
axonal projections from the CA3 region of the hippocampus
memories involved in navigation of our surroundings and the creation of a mental map of our world
A test commonly used on rodents to study spatial memory behaviorally in non-human animals
non-human behavioral test used to assess the capacity for learning navigational cues
brain structure contributing heavily to processing valence of emotional experiences
non-human test for emotional memory
a neutral stimulus is associated with a conditioned response that someone wants to avoid
one of the signaling pathways important for visual memories
a component of the inferotemporal cortex involved in facial recognition
a component of the inferotemporal cortex contributing to visual memories associated with locations and environmental scenes
used for such behaviors as motor and habit learning, emotional processing, and action selection
characterized by the presence of recurring, intrusive thoughts, which can lead to repetitive actions
a natural and healthy series of stereotyped actions that consists of licking the paws and moving them through the fur of the nose, caudally down the body
the ability for brain circuits to store some piece of information
process that enables more permanent memory storing
the specific circuit of neurons that represent a memory
the specific circuit of neurons that represent a memory
the process by which networks of neurons fire repeatedly; thought to be the mechanism that allows for consolidation of memories
the process in which stored memories are recalled
in the vocabulary recall test, a subject must recall as many words from a list as possible with no cues
in the vocabulary recall test, a subject must recall as many words from a list as possible after given the categories of the words
restoring of a memory after its retrieved
memories that are not true to reality
special population of pyramidal cells of the hippocampus contributing strongly to location and navigational memory
a series of cortical neurons in the temporal lobe that increase their firing exclusively in response to highly-specific stimuli
the ability to change over time
when synaptic strength is increased and remains elevated
when synaptic strength is decreased and remains weak
a temporary depolarization of neurons
an intense electrical stimulation where axons are stimulated at 100 Hz for multiple seconds
glutamate receptors that we generally imagine as contributing to excitation
similar to the ionotropic AMPA receptors because they are permeable to cations and therefore excitatory, but also contains an Mg2+ ion in the center and is permeable to Ca2+ ions
activated by Ca2+ ions and it's main molecular action is to phosphorylate proteins
an intracellular protein that regulates the expression of genes
detecting the occurrence of separate input signals
synapses that do not change their activity even with glutamate release
a defensive motor response behavior in Aplysia
the suppression of a normal reflex behavior that is dependent on LTD
a depolarization of the membrane of a single neuron
short for excitatory postsynaptic potential
a phenomenon opposite to tolerance: instead of a drug effect being lessened after chronic exposure, a sensitized person experiences an increase in drug effect
signaling molecules which are chemically similar to the primary psychoactive ingredient of Cannabis
inhibitory Gi/o coupled receptors that respond to the presence of eCBs
mechanism of plasticity where cannabinoid receptors inhibit cAMP signaling, decreasing release probability
atypical neurotransmitter that contributes to LTD by activating an intracellular receptor called soluble guanylate cyclase
an intracellular receptor that changes the activity of the signaling molecule cGMP, which can either lead to enhancement or depression of synaptic strength
plasticity occurring when the paired pulse ratio is greater than 1
plasticity occurring when the paired pulse ratio is less than 1
the time between the pulses
interevent interval
a value obtained by dividing the amplitude of the second EPSP by the amplitude of the first EPSP
channels on the membrane that open during depolarization and allows for pre-synaptic Ca2+ to enter
an irreversible, slowly progressing neurodegenerative condition that leads to deficits in thinking, behavior, and memory loss
Alzheimer's disease that's diagnosed when a person is in their 50s or 60s, and is strongly influenced by genetic risk factors
more common form of Alzheimer's disease that's believed to be caused by a combination of old age and environmental factors in addition to genetic risk factors
greatest genetic risk factor to Alzheimer's disease
suggests that the loss of cholinergic neurons and the loss of acetylcholine signaling is the main pathological driver of Alzheimer's Disease
drugs that act to increase levels of acetylcholine
plaques that can cause neuronal death and lead to the cognitive deficits observed in Alzheimer's
an intracellular pathological marker of Alzheimer's Disease and correlate strongly with cognitive deficits in Alzheimer's Disease
suggests that the main driving factor of Alzheimer's is the deposition of Aβ in the brain, and this in turn leads to neurodegeneration via cell death, abnormal protein buildup, and neuroinflammation
an integral membrane protein expressed by neurons
the major microtubule-associate protein of mature neurons, and helps to function to maintain cellular morphology. Excess phosphorylation of this protein causes tau to accumulate inside the cell, leading to neuronal dysfunction and cell death
a disorder resulting from a severe deficiency of thiamine
an essential vitamin that functions in metabolic processes
the fabrication of false memories ranging from subtle to wildly fantastical
paired structures located at the ventral surface of the brain close to the brain stem
injuries sustained by a sudden blow to the head
mild form of a traumatic brain injury
an injury where the brain hits the inside of the skull closest to the external force
an injury where the brain recoils backwards and hits the interior surface of the skull opposite of the cause of the insult